Thank you for visiting nature.com. You are using a browser version with limited support for CSS. To obtain the best experience, we recommend you use a more up to date browser (or turn off compatibility mode in Internet Explorer). In the meantime, to ensure continued support, we are displaying the site without styles and JavaScript.
- View all journals

Organometallic chemistry articles from across Nature Portfolio
Organometallic chemistry is the study of the synthesis, structure and reactivity of chemical compounds that contain metal-carbon bonds. These compounds are often used as homogeneous catalysts.
Related Subjects
- Chemical bonding
- Cross-coupling reactions
- Metathesis reactions
Latest Research and Reviews

Water-catalyzed iron-molybdenum carbyne formation in bimetallic acetylene transformation
Bioinspired Fe-Mo complexes are promising for the activation of small molecules, offering valuable insights into the interactions and transformations of unsaturated hydrocarbons with abundant metals. Here, the authors report the synthesis and mechanistic studies of FeMo carbyne complexes and unveil the pivotal role of H 2 O as a proton shuttle.
- Xiaofang Zhai
- Minghui Xue
- Wenguang Wang
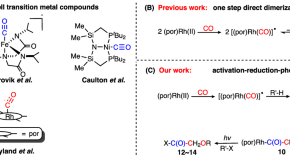
Photochemical conversion of CO to C1 and C2 products mediated by porphyrin rhodium(II) metallo-radical complexes
Reductive coupling of CO, a relatively inert molecule, enables its incorporation into both simple building block chemicals as well as more complex synthetic targets. Here, the authors exploit the reactivity of rhodium porphyrin complexes, using photoirradiative conditions to produce manifold reactive pathways after CO binding to the metal center.
- Jiajing Zhang

Structural and spectroscopic characterization of N 1 , N 2 -diphenylacenapthylene-1,2-diimines and the influence of substituents on the viscosity of alkyl magnesium solutions
- Julia Felicitas Schwarz
- Clemens Schwarzinger
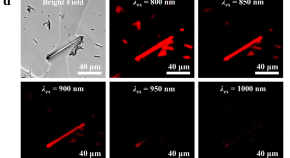
Unlocking multi-photon excited luminescence in pyrazolate trinuclear gold clusters for dynamic cell imaging
Coinage-metal-based cyclic trinuclear complexes have potential in diverse applications, but their use in biochemical applications has been limited due to particle size and hydrophobicity. Here, the authors report the development of such complexes with multi-photon luminescent properties for use in cell imaging.
- Yu-Xin Chen
- Gangfeng Ouyang
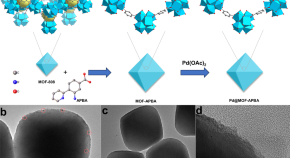
N, N’-bidentate ligand anchored palladium catalysts on MOFs for efficient Heck reaction
Metal-organic frameworks have earned attention for their potential to address the longevity of catalytic centers. Here, the authors have synthesized an amino-pyridine benzoic acid ligand to disperse palladium catalytic centers atomically resulting in a high average turnover number (95000) and low metal residue (4.8 ppm) in the Heck reaction.
- Nian-Nian Wang
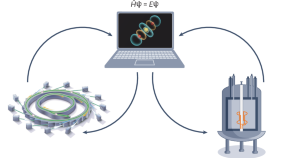
Understanding covalency in molecular f -block compounds from the synergy of spectroscopy and quantum chemistry
We describe recent advances in the understanding of covalency in the f element–other element bond through the synergistic application of computational quantum chemistry with nuclear magnetic resonance and X-ray spectroscopies.
- Nikolas Kaltsoyannis
- Andrew Kerridge
News and Comment
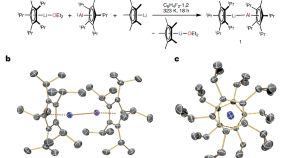
A molecular sandwich with a lithium–aluminium filling
Compounds containing metal–metal bonds can provide fresh insights into electronic structure and bonding, and their synthesis can open up new chemical space. A sandwich complex containing a lithium–aluminium bond has now delivered some food for thought in this arena.
- David P. Mills
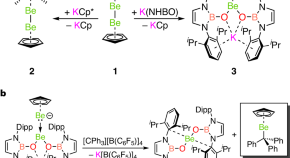
Polarizing the Be–Be bond
The Be–Be bond has long represented a ‘missing link’ between the H–H bond of dihydrogen and the B–B bond of diborane(4) species. Now, a complex with a polarized Be–Be bond that acts as a source of the beryllyl anion is reported. This work also reveals similarities between the homo-elemental linkages of boron and beryllium.
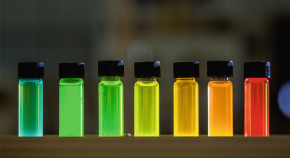
The synthesis behind the 2023 Nobel Prize
In 1993, a new route for the synthesis of semiconductor nanocrystals was reported that exploited organometallic chemistry to afford nearly monodisperse particles. 30 years later the award of the 2023 Nobel Prize in Chemistry can be directly traced to this single publication.
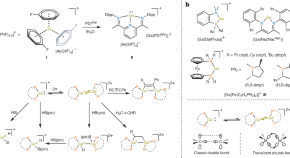
Catalytic reactions with low-valency ambiphilic gallium complexes
A one-pot method is introduced for synthesizing highly reactive and potentially chiral low-valency Ga( i ) complexes, facilitating their application as a versatile catalyst.
- Djamila Azrou
- Christophe Bour
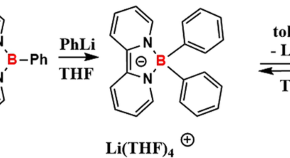
Borates expand their reduction power
The chemical reduction of group 1 metal cations to their zero-valent species is challenging. Now, a bipyridine-stabilized borate anion joins the ranks of suitable reducing agents and also proves active in the two-electron reduction of CO 2 .
- Johannes Kreutzer
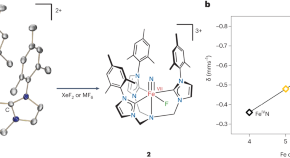
Lifting iron higher and higher
Biological and synthetic catalysts often utilize iron in high oxidation states (+IV and greater) to perform challenging molecular transformations. A coordination complex featuring an Fe(VII) ion has now been synthesized through sequential oxidations of nonheme iron–nitrido precursors.
- Adam T. Fiedler
- Laxmi Devkota
Quick links
- Explore articles by subject
- Guide to authors
- Editorial policies

Information
- Author Services
Initiatives
You are accessing a machine-readable page. In order to be human-readable, please install an RSS reader.
All articles published by MDPI are made immediately available worldwide under an open access license. No special permission is required to reuse all or part of the article published by MDPI, including figures and tables. For articles published under an open access Creative Common CC BY license, any part of the article may be reused without permission provided that the original article is clearly cited. For more information, please refer to https://www.mdpi.com/openaccess .
Feature papers represent the most advanced research with significant potential for high impact in the field. A Feature Paper should be a substantial original Article that involves several techniques or approaches, provides an outlook for future research directions and describes possible research applications.
Feature papers are submitted upon individual invitation or recommendation by the scientific editors and must receive positive feedback from the reviewers.
Editor’s Choice articles are based on recommendations by the scientific editors of MDPI journals from around the world. Editors select a small number of articles recently published in the journal that they believe will be particularly interesting to readers, or important in the respective research area. The aim is to provide a snapshot of some of the most exciting work published in the various research areas of the journal.
Original Submission Date Received: .
- Active Journals
- Find a Journal
- Proceedings Series
- For Authors
- For Reviewers
- For Editors
- For Librarians
- For Publishers
- For Societies
- For Conference Organizers
- Open Access Policy
- Institutional Open Access Program
- Special Issues Guidelines
- Editorial Process
- Research and Publication Ethics
- Article Processing Charges
- Testimonials
- Preprints.org
- SciProfiles
- Encyclopedia
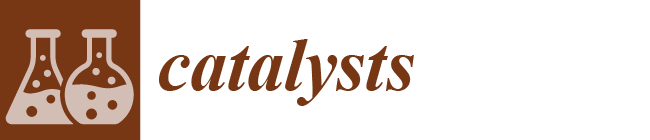
Article Menu
- Subscribe SciFeed
- Google Scholar
- on Google Scholar
- Table of Contents
Find support for a specific problem in the support section of our website.
Please let us know what you think of our products and services.
Visit our dedicated information section to learn more about MDPI.
JSmol Viewer
Recent advances in organometallic chemistry and catalysis.

Conflicts of Interest
- Jahnke, M.C.; Hahn, F.E. Chemistry of N-heterocyclic carbene ligands. Top. Organomet. Chem. 2010 , 30 , 95–129. [ Google Scholar ]
- Nolan, S.P. (Ed.) N-Heterocyclic Carbenes ; Wiley-VCH: Weinheim, Germany, 2014. [ Google Scholar ]
- Peris, E. Smart N-heterocyclic carbene ligands in catalysis. Chem. Rev. 2018 , 118 , 9988–10031. [ Google Scholar ] [ CrossRef ]
- Samojłowicz, C.; Bieniek, M.; Grela, K. Ruthenium-based olefin metathesis catalysts bearing N-heterocyclic carbene ligands. Chem. Rev. 2009 , 109 , 3708–3742. [ Google Scholar ] [ CrossRef ]
- Jolly, P.I.; Marczyk, A.; Małecki, P.; Trzybiński, D.; Woźniak, K.; Kajetanowicz, A.; Grela, K. Specialized olefin metathesis catalysts featuring unsymmetrical N -heterocyclic carbene ligands bearing N -(fluoren-9-yl) arm. Catalysts 2020 , 10 , 599. [ Google Scholar ] [ CrossRef ]
- Kantchev, A.B.; O’Brien, C.J.; Organ, M.G. Pd-N-heterocyclic carbene (NHC) catalysts for cross-coupling reactions. Aldrichchim. Acta 2006 , 39 , 97–111. [ Google Scholar ] [ CrossRef ]
- O’Brien, C.J.; Kantchev, E.A.B.; Valente, C.; Hadei, N.; Chass, G.A.; Lough, A.; Hopkinson, A.C.; Organ, M.G. Easily prepared air- and moisture-stable Pd-NHC (NHC = N-heterocyclic carbene) complexes: A reliable, user-friendly, highly active palladium precatalyst for the Suzuki-Miyaura reaction. Chem. Eur. J. 2006 , 12 , 4743–4748. [ Google Scholar ] [ CrossRef ]
- Valente, C.; Pompeo, M.; Sayah, M.; Organ, M.G. Carbon-heteroatom coupling using Pd-PEPPSI complexes. Org. Process Res. Dev. 2014 , 18 , 180–190. [ Google Scholar ] [ CrossRef ]
- Froese, R.D.J.; Lombardi, C.; Pompeo, M.; Rucker, R.P.; Organ, M.G. Designing Pd-N-heterocyclic carbene complexes for high reactivity and selectivity for cross-coupling applications. Acc. Chem. Res. 2017 , 50 , 2244–2253. [ Google Scholar ] [ CrossRef ] [ PubMed ]
- Al Nasr, I.; Touj, N.; Koko, W.; Khan, T.; Özdemir, I.; Yaşar, S.; Hamdi, N. Biological activities of NHC-Pd(II) complexes based on benzimidazolylidene N -heterocyclic carbene (NHC) ligands bearing aryl substituents. Catalysts 2020 , 10 , 1190. [ Google Scholar ] [ CrossRef ]
- Ndagi, U.; Mhlongo, N.; Soliman, M.E. Metal complexes in cancer therapy—An update from drug design perspective. Drug Des. Devel. Ther. 2017 , 11 , 599–616. [ Google Scholar ] [ CrossRef ] [ Green Version ]
- Chylewska, A.; Biedulska, M.; Sumczynski, P.; Makowski, M. Metallopharmaceuticals in therapy—A new horizon for scientific research. Curr. Med. Chem. 2018 , 25 , 1729–1791. [ Google Scholar ] [ CrossRef ]
- Fukuda, Y.; Utimoto, K. Effective transformation of unactivated alkynes into ketones or acetals by means of Au(III) catalyst. J. Org. Chem. 1991 , 56 , 3729–3731. [ Google Scholar ] [ CrossRef ]
- Hashmi, A.S.K.; Toste, F.D. (Eds.) Modern Gold Catalyzed Synthesis ; Wiley-VCH: Weinheim, Germany, 2012. [ Google Scholar ]
- Toste, F.D.; Michelet, V. (Eds.) Gold Catalysis: An Homogeneous Approach ; Imperial College Press: London, UK, 2014. [ Google Scholar ]
- Cadierno, V. Gold-catalyzed addition of carboxylic acids to alkynes and allenes: Valuable tools for organic synthesis. Catalysts 2020 , 10 , 1206. [ Google Scholar ] [ CrossRef ]
- Cadierno, V. Metal-catalyzed synthesis and transformations of β -haloenol esters. Catalysts 2020 , 10 , 399. [ Google Scholar ] [ CrossRef ] [ Green Version ]
- Wu, W.; Jiang, H. Haloalkynes: A powerful and versatile building block in organic synthesis. Acc. Chem. Res. 2014 , 47 , 2483–2504. [ Google Scholar ] [ CrossRef ] [ PubMed ]
- Cadierno, V. Metal-catalyzed hydrofunctionalization reactions of haloalkynes. Eur. J. Inorg. Chem. 2020 , 2020 , 886–898. [ Google Scholar ] [ CrossRef ]
- Davenel, V.; Nisole, C.; Fontaine-Vive, F.; Fourquez, J.-M.; Chollet, A.-M.; Michelet, V. Gold-catalyzed cycloisomerization of 1,6-cyclohexenylalkyne: An efficient entry to bicyclo[3.2.1]oct-2-ene and bicyclo[3.3.1]nonadiene. J. Org. Chem. 2020 , 85 , 12657–12669. [ Google Scholar ] [ CrossRef ]
- Davenel, V.; Puteaux, C.; Nisole, C.; Fontaine-Vive, F.; Fourquez, J.-M.; Michelet, V. Indium-catalyzed cycloisomerization of 1,6-cyclohexenylalkynes. Catalysts 2021 , 11 , 546. [ Google Scholar ] [ CrossRef ]
- Benaglia, M. (Ed.) Recoverable and Recyclable Catalysts ; John Wiley & Sons: Chichester, UK, 2009. [ Google Scholar ]
- Caballero, V.; Estevez, R.; Luna, D.; Bautista, F.M.; Romero, A.A.; Aguado-Deblas, L.; Hidalgo-Carrillo, J.; Romero, I. Hydrogenation of α , β -unsaturated carbonyl compounds over covalently heterogenized Ru(II) diphosphine complexes on AlPO 4 -sepiolite supports. Catalysts 2021 , 11 , 289. [ Google Scholar ] [ CrossRef ]
- Ribeiro, A.P.C.; Spada, E.; Bertani, R.; Martins, L.M.D.R.S. Adipic acid route: Oxidation of cyclohexene vs. cyclohexane. Catalysts 2020 , 10 , 1443. [ Google Scholar ] [ CrossRef ]
- Castellan, A.; Bart, J.C.J.; Cavallaro, S. Industrial production and uses of adipic acid. Catal. Today 1991 , 9 , 237–254. [ Google Scholar ] [ CrossRef ]
- Van de Vyver, S.; Román-Leshkov, Y. Emerging catalytic processes for the production of adipic acid. Catal. Sci. Technol. 2013 , 3 , 1465–1479. [ Google Scholar ] [ CrossRef ] [ Green Version ]
MDPI stays neutral with regard to jurisdictional claims in published maps and institutional affiliations. |
Share and Cite
Cadierno, V. Recent Advances in Organometallic Chemistry and Catalysis. Catalysts 2021 , 11 , 646. https://doi.org/10.3390/catal11050646
Cadierno V. Recent Advances in Organometallic Chemistry and Catalysis. Catalysts . 2021; 11(5):646. https://doi.org/10.3390/catal11050646
Cadierno, Victorio. 2021. "Recent Advances in Organometallic Chemistry and Catalysis" Catalysts 11, no. 5: 646. https://doi.org/10.3390/catal11050646
Article Metrics
Article access statistics, further information, mdpi initiatives, follow mdpi.
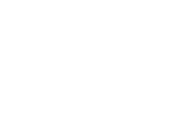
Subscribe to receive issue release notifications and newsletters from MDPI journals
- Utility Menu

gA4 tracking code
Department of chemistry and chemical biology.
- Donate to CCB
Organometallics
Studies in organometallic chemistry in CCB involve the design of new transition metal complexes that display specific, targeted functionality such as electron-transfer activity, redox behavior, or variable metal-metal interactions. Such complexes can function as models for biological metal-containing sites or as industrial catalysts, as examples.
Organometallics Faculty


Theodore Betley

Eric Jacobsen

Jarad Mason

Daniel G. Nocera
Recent news.

CCB celebrates 2024 Pathways Program Interns

Dan Kahne appointed Chair of the Department of Chemistry and Chemical Biology

Two CCB Postdocs awarded 2024 Beckman Postdoctoral Fellowships
Browse by research area.
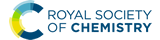
Organometallic catalysis in aqueous and biological environments: harnessing the power of metal carbenes

First published on 16th May 2022
Translating the power of transition metal catalysis to the native habitats of enzymes can significantly expand the possibilities of interrogating or manipulating natural biological systems, including living cells and organisms. This is especially relevant for organometallic reactions that have shown great potential in the field of organic synthesis, like the metal-catalyzed transfer of carbenes. While, at first sight, performing metal carbene chemistry in aqueous solvents, and especially in biologically relevant mixtures, does not seem obvious, in recent years there has been a growing number of reports demonstrating the feasibility of the task. Either using small molecule metal catalysts or artificial metalloenzymes, a number of carbene transfer reactions that tolerate aqueous and biorelevant media are being developed. This review intends to summarize the most relevant contributions, and establish the state of the art in this emerging research field.
1. Introduction
However, there has been an increasing demonstration that many organometallic complexes and intermediates do tolerate the presence of water. Indeed, a huge number of water-compatible organometallic reactions have been recently developed. 2 Work in the field has been further impelled by the growing interest in developing sustainable synthetic technologies, and by the observation that the presence of water can even accelerate some reactions. 3
These advances have led scientists to wonder if organometallic reactions could be carried out in biological and even living settings, as this could open new opportunities in cell biology and biomedicine. However, the translation of organometallic chemistry to biological habitats is not straightforward, and presents important challenges. First, a cellular milieu cannot be equated to a typical aqueous solvent, owing to its gel-like nature and intrinsic crowded environment. Furthermore, the presence of numerous biological components can promote the deactivation of the metal reagents or inhibit the catalytic cycles. Moving to living environments is further hampered by potential toxicity and transport issues, and by the low concentration of reagents and reactants.
Nonetheless, with the advent of bioorthogonal chemistry, as coined by Bertozzi and coworkers, 4 an increasing number of organic reactions, including metal-catalyzed processes, have been demonstrated to work in biological and living environments. 5,6 A key discovery that sparked the field is the well-known copper-catalyzed azide alkyne cycloaddition (CuAAC), paradigm of click chemistry, which is usually carried out with Cu( II ) reagents and external reducing agents like ascorbate. Despite the intrinsic toxicity of these reagents, an appropriate tuning of the reaction conditions has allowed its use in cellular settings. 7–11 Its impressive chemoselectivity has also led to many applications for bioconjugation and post-translational modification of proteins or nucleic acids. 12,13
The CuAAC entails a typical organometallic mechanism involving oxidative cyclometallation and reductive elimination steps, and it is a fundamental reference in the field of biological organometallic catalysis. Another early (2006) report that has gained increasing recognition by the chemical biology community is the discovery by Meggers et al. that Ru( II ) catalysts can promote the removal of alloc protecting groups in designed substrates, even in the presence of HeLa cells. 14
Although for several years there were not many new contributions in the area, in the last decade there has been an upsurge in reports dealing with transition metal-promoted reactions in biological media, and also in living organisms like bacteria. 15–20 Some of these discoveries have already found very relevant biological applications. 21–24 The reactions include uncaging processes, mainly those catalyzed by ruthenium or palladium complexes, such as the cleavage of N -allylcarbamates or O -allyl/propargyl ethers, 14,25–33 ruthenium catalyzed bond-forming reactions (annulations between thioalkynes and azides (RuAtAC), 34,35 (2 + 2 + 2) cycloadditions 36 ) or isomerization of allylic alcohols, 37 gold-catalyzed hydroarylations, 38 and even palladium promoted Sonogashira 39 or Suzuki–Miyaura 40–43 cross couplings.
Therefore, a wide range of organometallic transformations, from deprotection to cross-coupling, cyclization or cycloaddition reactions, have been demonstrated to be compatible with biological environments, provided appropriate metal complexes and probes are employed.
What about metal carbenes? Can their reactivity be exported to biological environments? Metal carbene transfer reactions are among the most powerful and versatile transformations in catalysis and organometallic chemistry, and have been used for many synthetic applications. 44,45 Translating the rich reactivity typically exhibited by metal carbenes in organic solvents to aqueous and biological media, could open important new avenues in research at the interface between chemistry and biology.
Indeed, in the last decade, there has been an increase in the number of reactions involving bioorthogonal metal-carbene transfer processes. In some cases, it has also been demonstrated that this chemistry can be performed in cells or in bacteria. In this review, we intend to cover the most significant contributions in this topic, from bioconjugations and chemoselective modifications of biopolymers to synthetic transformations of small molecules in biological settings, including bacteria or mammalian cells.
2. Catalysis involving metal carbene transfer reactions
(a) Electronic structure of carbenes. (b) Classification of metal carbenes based on the nature of the substituents adjacent to the carbene. (c) Some transformations of metal carbenes carried out in aqueous media. EDG = electron donating group; EWG = electron withdrawing group. |
A wide range of precursors have been employed for the generation of carbenes, such as tosylhydrazones, diaziridines, triazoles, cycloheptatrienes, alkynes or ynamides, among others. 47 However, the most widely used carbene precursors are diazo compounds, which are reasonably stable but can readily decompose to carbenes under mild reaction conditions. 44,48 The resulting free carbenes tend to be highly reactive, and therefore difficult to use in chemical transformations; however, upon appropriate coordination to transition metals they give metal carbenes, which present a more controllable and rich reactivity. The chemical properties of these metal complexes depend on the spin configurations of the carbene, and on the overlap with the metal orbitals.
Different criteria have been used to classify metal carbenes. Historically, they have been categorized as Fischer and Schrock carbenes, depending on the metal complex and the type of substituents at the carbene; but in the context of this review, it is useful a classification based on the nature of the substituents adjacent to the reactive carbene centre. Acceptor/acceptor (A/A) and acceptor (A) carbenes are highly reactive due to the lack of stabilization of the electrophilic carbene centre. In contrast, the presence of donor groups stabilizes the metal carbene centre and attenuates the reactivity. This is the case of donor/acceptor (D/A) carbenes, which have been widely used due to their good balance between reactivity and stability ( Fig. 1b ). 49,50
The rich reactivity of metal carbenes is mostly related to the charge distribution along the metal–carbon bond and the electrophilicity of the carbene carbon, allowing its reaction with a variety of nucleophiles. Within the broad spectrum of reactions enabled by metal carbenes, most common transformations include cyclopropanations of alkenes, 51–53 C–H functionalizations, 49,54–56 insertions into X–H bonds (X = O, N, S, Si), 57,58 the formation of ylides 59–61 (and subsequent rearrangements) or cycloaddition processes 44,61 ( Fig. 1c ). This reactivity is different from that exhibited by carbene precursors, usually diazocarbonyl compounds, which tend to decompose and/or engage in rearrangement or dimerization reactions.
The reactions of metal carbenes with substrates exhibiting non-polar bonds ( i.e. cyclopropanation of alkenes or insertions into C–H or Si–H bonds), tend to proceed through a concerted mechanism, 62 while in the case of insertions into polar X–H bonds a stepwise ylide mechanism might be operative. Beyond these general features, the characteristics of the transition metal complex play a key role in the mechanistic pathway. 58
Transition metal-catalyzed carbene transformations have been well studied in organic solvents, with rhodium, copper and iron being the most explored metals. It is important to note that besides carbene transfer reactions from diazo or related precursors, metal carbenes can also be used as catalysts, e.g. in metathesis processes.
3. Metal-promoted carbene transfer reactions in aqueous media
3.1. transformations of small molecules.
Metal catalyzed cyclopropanations. Top: General reaction scheme. Bottom-left: Asymmetric Ru-catalyzed cyclopropanation reported by Nishiyama. Bottom-right: Rh-catalyzed cyclopropanation reported by Charette and Wurz. |
In 2002 Charette and Wurz reported a different approach to perform similar cyclopropanations. They found that the combination of hydrophobic rhodium catalysts such as dirhodium( II ) octanoate (Rh 2 (Oct) 4 ) with hydrophobic alkenes allows the formation of “small alkene/catalyst beads or micelles” in water. Then, a slow diffusion of EDA through the surface of the beads enabled a controlled formation of the desired cyclopropanated products in high yields ( Fig. 2 ). Noticeably, water-soluble rhodium carboxylates (Rh 2 (OAc) 4 or Rh 2 (O 2 CCF 3 ) 4 ) were inefficient, leading to low yields. An asymmetric version of this reaction was also studied using ruthenium( II ) and cobalt( II ) chiral catalysts. 65
A family of catalysts that has been extensively employed for cyclopropanation reactions in organic solvents are metalloporphyrins, an interesting type of metal complexes that is also present in certain metalloenzymes with oxidative functions. In 2008, Simonneaux and coworkers prepared water-soluble ruthenium and iron porphyrins by the introduction of sulfonate groups, and used them as catalysts for the asymmetric cyclopropanation of styrene in water, with EDA as the carbene precursor ( Fig. 3a ). They obtained the desired products in yields up to 85%, with high diastereoselectivity ( trans / cis up to 96/4) and good enantioselectivity for the trans isomer (83%). 66
(a) Water-soluble Fe and Ru porphyrins developed by Simonneaux and coworkers for asymmetric cyclopropanations in water. (b) Cobalt porphyrin “ship in a bottle” developed by de Bruin and coworkers, reproduced from ref. with permission from Wiley-VCH GmbH, copyright 2014. |
Another interesting approach based on the use of nanoreactors was reported in 2014 by van Hest et al. , who immobilized chiral bis(oxazoline)–copper catalysts inside a polymersome membrane to perform asymmetric cyclopropanations of alkenes in water. Interestingly, only hydrophobic alkenes underwent the cyclopropanation, likely because of their ability to localize into the active site of the polymer. 68
All these results confirm the compatibility of specific metal carbenes with water, which even plays an important role in the reactivity and selectivity of the processes by eliciting hydrophobic effects.
C–H insertion reactions in water. (a) Intramolecular insertion of rhodium carbenoids into aliphatic C–H bonds reported by Afonso and coworkers. (b) Functionalization of indoles and anilines by in situ generated α-oxo gold carbenes. |
Further studies revealed that the use of more hydrophobic catalysts such as Rh 2 (Oct) 4 partially avoided or completely suppressed the hydroxylation reaction, even when less hydrophobic substrates were employed. 70
Another interesting metal-carbene mediated C–H insertion was proposed by the group of Ye, using ynamides as carbene precursors and gold catalysts. In the presence of an oxidant, the reaction generates α-oxo gold carbenes that can be trapped by indoles or anilines ( Fig. 4b ). The intermolecular reactions were carried out at 80 °C using water as reaction media, providing the C-alkylation products in excellent yields, after 1–2 hours. 71 Remarkably, the presence of water suppressed undesired over-oxidations of the gold carbene.
N–H insertion reactions in water. (a) N–H insertion of iron carbenoids into amino acid derivatives catalyzed by a water soluble iron porphyrin in citrate buffer (CBS) reported by Simonneaux. (b) Carbene insertions into N–H bonds of anilines catalyzed by copper or iridium complexes reported by Sivasankar. (c) Iron catalyzed annulation of 1,2-diamines and diazodicarbonyl compounds reported by Lee. |
In 2016, Sivasankar and coworkers reported an efficient synthesis of α-amino phosphonates in water via insertion of copper( I ) carbenoids into the N–H bond of anilines under mild reaction conditions ( Fig. 5b ). The best results were obtained with (CH 3 CN) 4 CuClO 4 as catalyst, yielding the desired N–H insertion products in short reaction times (from 15 min to 2 h in most cases). 73 Later on, in 2017, they found conditions for the N–H insertion of carbenes exhibiting different electronic properties. AgOTf was the best choice for donor/donor carbene precursors, providing moderate yields after 5 minutes of reaction, while for donor/acceptor carbenes containing aryl and ester groups, Pd 2 (dba) 3 led to the product in 90% yield after 30 minutes. In the case of acceptor/acceptor carbenes, the most efficient catalyst was found to be [Ir(COD)Cl] 2 , affording the N–H insertion product in 81% yield after 12 h. The authors studied in more detail the reaction for acceptor/acceptor carbenes, and used their method to synthesize a series of aniline-derivatives in good yields. However, in the case of aliphatic amines, such as benzylamine, and heteroaromatic amines like 2-aminopyridine, only traces amounts of the N–H insertion products were detected. 74
In 2016, Lee and coworkers reported an elegant strategy for the synthesis of quinoxalines, pyrazines and benzoquinoxalines through the annulation of 1,2-diamines and diazodicarbonyls in water at 70 °C, using Fe(OTf) 3 as catalyst ( Fig. 5c ). The process is initiated by the insertion of an iron carbenoid into the N–H bond of the diamine, followed by cyclization and oxidative aromatization, to afford the desired heterocycles in excellent yields. 75
Gross and coworkers reported N–H insertion reactions of EDA and ethyl diazopropionate (EDP) on anilines in aqueous solutions, catalyzed by iron corrole conjugated albumins. The authors did not observe enantiomerically enriched products in any of the cases, and did not comment on the potential accelerating effect of the protein. 77
(a) Gold-catalyzed tandem O–H insertion/cyclization in DMF/H O. (b) Doyle–Kirmse reaction of in situ generated sulfonium ylides. |
3.2. Chemoselective reactions of peptides and proteins
(a) Bioconjugation of peptides and proteins based on the selective alkylation of tryptophan residues using rhodium carbenoids reported by Antos and Francis. (b) Bioconjugation of proteins based on the alkylation of the N-terminus. (c) Bioconjugation using Fischer carbenes on gold or glass surfaces. (d) Proximity-driven bioconjugation using rhodium metallopeptides developed by Ball for the site-specific modification of peptides, proteins and antibodies. The figure shows the structure of subtilisin Carlsberg ((a) PDB ID: , tryptophans in blue) and RNase A ((b) PDB ID: , N-terminus in blue). |
In 2013 Ball demonstrated the feasibility of performing selective cysteine modification under mild reaction conditions, using a N -( tert -butyl)hydroxylamine-containing buffer employed by Antos and Francis, Rh 2 (OAc) 4 and a biotin-tethered diazo substrate. The authors applied these reaction conditions to a mixture of proteins, and observed the modification of proteins with accessible cysteine thiols, while those with buried or oxidized cysteine residues remained unmodified. 84
Che et al. reported the first example of a metalloporphyrin-catalyzed carbene transfer for the selective modification of proteins. A designed water-soluble ruthenium glycosylated porphyrin catalyst [Ru II (4-Glc-TPP)(CO)] (1,4-Glc-TPP = meso-tetrakis(4-(β- D -glucosyl)phenyl)porphyrinato dianion) was employed for different carbene transfer reactions in aqueous media (inter- and intramolecular cyclopropanation, Doyle–Kirmse reaction, N–H insertion). Interestingly, the catalyst proved to be effective for the selective alkylation of the N-terminus of peptides and proteins using a fluorescent-tethered diazo compound ( Fig. 7b ). However, when the protein presented free thiols (cysteines) in its structure, the insertion of the metal carbene took place into the S–H bond rather than in the terminal N–H bond. 85
Despite these successes, the reactions tend to be low yielding, and chemoselectivities are, in many cases, modest. As an alternative to these methods, Ball and coworkers developed an elegant strategy based on proximity-driven bioconjugations that combines molecular recognition with metallocarbene reactivity. This approach is based on the use of a dirhodium metallopeptide made of a rhodium( II ) catalyst conjugated to a peptide that is able to recognize another specific protein ( via a coiled-coil assembly, Fig. 7d ). 88
Using this approach, the authors performed site-specific modification of peptides and proteins at physiological pH, and in biologically relevant buffers. 89–91 The use of biotin–diazo conjugates as reagents allowed for affinity tagging of the target proteins. They were even able to use designed metallopeptide catalysts to perform selective protein modifications in E. coli lysate. Moreover, they developed a strategy for the site-specific functionalization of antibodies based on the use of a hexarhodium metallopeptide catalyst with affinity for the Fc fragment of the antibody. 92 Based on molecular recognition of the Fc region, this approach enabled the introduction of orthogonal alkyne handles into mono- or polyclonal antibodies, opening the door to the quick production of antibody conjugates.
3.3. Chemoselective modification of nucleic acids
Nucleic acid alkylation using metal carbenes. (a) Structure-selective catalytic alkylation of DNA and RNA (left) and tandem carbene N–H insertion/CuAAC (right) reported by Gillingham. (b) Chemoselective alkylation at guanine O -G using copper carbenes reported by Gillingham. (c) Site-selective functionalization of oligonucleotides using rhodium carbenes reported by Park, reproduced with permission from ref. , licensed under a Creative Commons Attribution (CC BY) license. It is attributed to Park, and the original version can be found here (https://www.nature.com/articles/s41467-021-21839-4). |
Shortly thereafter, they demonstrated the effectiveness of Cu( I ) salts, generated from copper sulfate and sodium ascorbate, to catalyze a similar insertion of donor/acceptor carbenes into the N–H bond of adenine in MES buffer. The diazo compound was further modified to contain an alkyne or azide handle, thus developing a tandem carbene insertion/CuAAC reaction ( Fig. 8a , right). 94
Interestingly, the use of acceptor carbenes generated from α-diazoesters or diazoacetamides and copper( I ) led to chemoselective alkylation of the O 6 position in guanine (O 6 -G) in mono- and oligonucleotides, attributed to the pre-coordination of the catalyst to the N 7 of guanine ( Fig. 8b ). 95 However, with complex oligonucleotides containing multiple chelation sites, reaction rates are low, likely due to unproductive catalyst sequestration.
Very recently, Park and coworkers reported an elegant site-selective post-synthetic modification of oligonucleotides at unpaired guanosines. The use of a coordinatively saturated Rh( I ) catalyst like [Rh(COD)Cl] 2 circumvented the chelation issues related to the deactivation of the catalyst observed by Gillingham, while maintaining the chemoselectivity towards base-unpaired guanosines in single- and double-stranded oligonucleotides. They exploited this feature and introduced guanosine-bulge loops in duplexes, which resulted in high regioselectivity, and allowed iterative introduction of multiple modifications in a programmable fashion ( Fig. 8c ). 96 The utility of this approach was further showcased by performing DNA-protein cross-linking in cell lysate.
4. Carbene transfer reactions catalyzed by metallobiopolymers
The design and development of ArMs has been largely based on repurposing natural metalloenzymes or on anchoring a metal cofactor to a protein-binding pocket. In the first approach ( Fig. 9 , left), a natural metalloenzyme is modified by directed mutagenesis and/or by introducing a non-natural metal-containing cofactor. In the second approach ( Fig. 9 , right), the metal can be either covalently bound to protein residues, or anchored into a protein cavity through supramolecular association ( e.g. biotin-modified catalysts in streptavidin, strategy primarily developed by Ward).
Main strategies for the development of ArMs. |
Although the overwhelming number of reports of the use of ArMs to perform carbene transfer reactions cannot be covered in this review, we present some selected contributions. In this first section, we will mainly focus on the strategies employed to prepare new ArMs and their reactivity profiles, while the following section spotlights the compatibility of the systems with living cells. Besides, we will also disclose some examples of the use of nucleic acids instead of protein as coatings for the catalytic metals in carbene transfer processes.
4.1. Reactions promoted by heme-containing artificial metalloenzymes
Arnold and coworkers, envisioning the structural similarity between the iron-oxo intermediate involved in cytochrome P450-catalyzed oxidations and an iron carbene, repurposed the system to work as a carbene transferase. In 2013, using as benchmark reaction the cyclopropanation of styrene with EDA, they demonstrated that variants of P450 BM3 from Bacillus megaterium can promote the carbene transfer, forming cyclopropane products with high levels of diastereo- and enantioselectivity. 104,105 Additional studies using directed evolution to introduce mutations at the binding pocket of different P450 cytochrome variants allowed to tune the selectivity and activity. Therefore, in the last decade, the group has successfully engineered new variants that catalyze cyclopropanation 106,107 and cyclopropenation 108 reactions, as well as N–H, 109 Si–H, 110 B–H 111 and C–H 112,113 insertions, with high rates and turnovers, and exquisite regio- and stereocontrol.
In 2014, Fasan et al. extended this strategy to other heme proteins, developing evolved variants of myoglobin (Mb) and horseradish peroxidase. Furthermore, they demonstrated that it is possible to replace the heme group by porphyrin cofactors with abiotic metals ( i.e. Mn or Co), to give metalloenzymes with similar levels of activity and selectivity. 114 Using directed evolution, they reported Mb mutants that successfully catalyze the cyclopropanation of a variety of aryl-substituted olefins with EDA, with excellent levels of diastereo- and enantioselectivity. 115–120 They also developed variants that catalyze carbene transfer reactions such as insertions into N–H, 121–123 S–H 124 or Si–H 125 bonds, and ylide formations. 126
Insertion of carbenes into C(sp )–H bonds catalyzed by several metal–PIX reconstituted myoglobins containing a single mutation at the axial residue. |
It is also possible to build ArMs with modified heme scaffolds, such as the one developed by Lehnert with mesoporphyrin IX (RuMpIX) incorporated into wild-type (wt) Mb and Mb mutants ( Fig. 11 ). However, these modified proteins exhibited moderate catalytic activity towards the cyclopropanation of styrene derivatives, and in N–H modifications of aniline derivatives. 130 Hayashi et al. selected an iron porphycene cofactor to reconstitute myoglobin, and demonstrated that the modified protein significantly accelerates a catalytic cyclopropanation reaction, which turned to be 615-fold faster than that obtained using native myoglobin ( Fig. 11 ). Interestingly, the authors performed mechanistic studies and reported the first spectroscopic observation of the active metallocarbene species in this type of reconstituted proteins. 131
Schematic representation of the reconstitution of myoglobin with RuMpIX and FePc. rMb = reconstituted myoglobin. |
All these impressive contributions demonstrate the enormous power of ArMs to perform carbene transfer reactions in aqueous buffers, with rates and selectivities much better than those achieved under standard organometallic conditions with discrete catalysts.
De novo designed metalloenzymes. (a) Rhodium ArM developed by Lewis. (b) Self-assembled LmrR-heme metalloenzyme reported by Roelfes, reproduced with permission from ref. , licensed under a Creative Commons Attribution (CC BY-NC 4.0) license, by Roelfes. Discolored from original. The original version can be found here (https://onlinelibrary.wiley.com/doi/full/10.1002/anie.201802946). (c) Streptavidin-biotin strategy followed by Ward. |
In 2018, Roelfes and coworkers reported the de novo design of a non-native heme protein based on the large, promiscuous, hydrophobic binding pocket of the lactococcal multidrug resistance regulator (LmrR) ( Fig. 12b ). By self-assembly of hemin and LmrR in a buffered solution, they created a biocatalyst for the cyclopropanation of styrene derivatives with EDA. The reaction requires an inert atmosphere and the addition of sodium dithionate as a reductant agent to generate the active Fe( II ) heme complex. Among the different mutants synthesized, LmrR_M8A delivered the product with good yield (45%) and reasonable enantiomeric excess (ee = 51% [1 R ,2 R enantiomer]), and TTNs up to 449. Interestingly, the crystal structure showed that the iron centre is apparently inaccessible for the substrates, as it is sandwiched between two tryptophan residues, and therefore, the dynamic nature of the enzyme seems to be responsible for the observed activity and enantioselectivity. 135
Inspired by the work of Wilson and Whitesides, 136 several groups, and especially Ward's, have designed artificial enzymes based on the use of biotin–avidin interactions to tether the metal cofactor to the protein. In 2018, the group demonstrated that covalently anchoring a dirhodium catalyst to a biotin, and embedding it within engineered streptavidin, gives a hybrid catalyst for olefin cyclopropanation and carbene C–H insertion reactions, albeit with modest performances ( Fig. 12c ). 137 Importantly, they demonstrated that the strategy can be implemented to work in the periplasm of E. coli ( vide infra ).
4.2. Carbene transformations promoted by metals coated on DNA scaffolds
Pioneering work in this field of DNA-based organometallic catalysis was performed by Roelfes et al. ( Fig. 13a ). Using copper complexes with intercalating ligands (dppz derivatives), and salmon testes duplex DNA as a source of chirality, they achieved asymmetric intramolecular cyclopropanation of α-diazo-β-ketosulfones, with enantiomeric excesses up to 84%. 138 Likely, the microenvironment resulting from the stacking of the ligands between the DNA bases may favour cyclopropanation over water addition to the carbene.
DNA-based carbene transfer reactions. (a) Intramolecular cyclopropanation catalyzed by assembled DNA/Cu complexes. (b) Cyclopropanation of styrene with EDA using DNA-based biocatalysts. |
They also implemented the use of porphyrin metal ligands in this type of carbene transfer reactions. In 2016 they reported a DNA-accelerated cyclopropanation of styrene derivatives with EDA, with a moderate enantiomeric excess of 53% in the presence of the salmon testes DNA/cationic iron porphyrin hybrid catalyst ( Fig. 13b ). An ortho -substituted N -methylpyridinium porphyrin that predominantly interacts with DNA through groove binding, and makes the catalytic site accessible for substrates, was shown to be the best catalyst. 139 The authors proposed that the rate acceleration observed may be related to the formation of hydrophobic pockets in the DNA upon binding of the iron porphyrin, thus providing a high effective molarity that benefits reactivity.
Li et al. built hybrid systems using intramolecular antiparallel G-quadruplex variants of thrombin-binding aptamer (TBA) G4-DNAs and cationic Fe porphyrin FeTMPyP4 (Fe-meso-tetra( N -methyl-4-pyridyl)porphyrin, Fig. 13b ). After adequate mutations, two efficient stereocomplementary catalysts were identified. Specific residues in the G4 structure, spatially close to the active site, were crucial for obtaining good selectivities in cyclopropanation reactions. 141
5. Exporting metal carbene reactions into biological environments and living cells
5.1. organometallic reactions in living contexts.
As indicated in the previous sections, reactions involving metal carbenes can be performed in aqueous media, using specifically tailored conditions. A more challenging issue is related with their bioorthogonality, and the possibility of exporting them to complex biological environments and living settings. Although advances in this area have been scarce, a number of reports increasingly show the potential of metal-promoted carbene transfer reactions in the fields of bioorthogonal and biological chemistry.
5.2. Carbene transfer reactions in cellular settings and in bacteria
Ru-catalyzed fusion of a diazo with an enyne in complex biological media to provide bicyclic cyclopropanes reported by Teply and coworkers. |
A particularly remarkable contribution in the area was disclosed by Balskus et al. , who demonstrated that metallocarbene chemistry can be interfaced with bacterial metabolism. They engineered E. coli to generate styrene from D -glucose, and integrated metallocarbene intermediates into the metabolic pathway using a biocompatible iron( III ) phthalocyanine catalyst (FePcCl) that transformed styrene into non-natural phenyl cyclopropanes in single-vessel fermentations ( Fig. 15 ). 143
Combination of microbial metabolism and metallocarbene chemistry to generate cyclopropanes from D-glucose in E. coli reported by Balskus. |
Very recently, Mascareñas and coworkers reported the first example of a metal-carbene transfer reaction within live mammalian cells ( Fig. 16 ). Using a common copper salt (Cu(OAc) 2 ), they synthesized benzoquinoxalines in cellulo , in a one-pot process that is initiated by an N–H carbene insertion of the in situ generated copper carbenoid into ortho -amino arylamines. The intracellular generation of a fluorescent benzoquinoxaline allowed for in vivo monitoring of the reaction by fluorescence microscopy. Remarkably, the methodology could be applied to elicit biological effects through the intracellular synthesis of Tyrphostin AG1385, a tyrosine phosphorylation inhibitor that disrupts mitochondrial functions, producing mitochondrial fragmentation and depolarization. Moreover, the authors were able to elicit cell-selective responses by equipping the copper catalyst with a targeting unit (RGD motif). 144
Exporting metal carbenes to live mammalian cells. Copper catalyzed synthesis of quinoxalines enabled by N–H carbene insertion. |
Comparison of Fe-oxene and Fe-carbene intermediates in heme-based metalloenzymes proposed by Arnold. |
It is worth noting the strategy reported by Arnold in 2016, which allowed the in vivo production of organosilicon compounds ( Fig. 18a ). 110 In this case, cytochrome c from Rhodothermus marinus (Rma cyt c) was shown to be the most active hemoprotein. Importantly, high levels of selectivity were achieved for Si–H carbene insertions. Even in the presence of other functionalities such as alcohols or primary amines, styryl olefins or electron rich double bonds, susceptible of undergoing O–H, N–H insertion or cyclopropanations, the C–Si bond formation was predominant. Interestingly, by selecting the adequate ArMs, the authors were able to selectively catalyze the insertion of the carbene either into a Si–H or N–H bond of the same substrate ( Fig. 18b ). 145
Expanding nature's carbene catalytic repertoire for in vivo (E. coli) reactions. (a) Synthesis of chiral silicon compounds. (b) Chemoselectivity of carbene transfer: Si–H versus N–H insertion, reproduced from ref. with permission from American Chemical Society, copyright 2021. (c) Synthesis of chiral boron compounds. |
Using directed evolution, they could enhance the catalytic function of this ArM for B–H insertions, reporting, one year later, a fully genetically encoded platform for producing chiral organoboranes in bacteria ( Fig. 18c ). 111 These enzymes were found to form carbon–boron bonds in the presence of borane–Lewis base complexes, through carbene insertion into the boron–hydrogen bond. Surprisingly, the variants demonstrated to be even more productive when the reactions were carried out using whole Escherichia coli cells expressing this enzyme.
The P411 scaffold has been further optimized by directed evolution, and demonstrated to work in E. coli for other reactions, such as the synthesis of bicyclobutanes, via successive carbene addition to unsaturated carbon–carbon bonds. 146
Fasan and coworkers reported the first example of intramolecular olefin cyclopropanations with allyl α-diazoacetate derivatives (with high stereocontrol and complementary enantioselectivities), in whole cells, using engineered Mb based catalysts. 147 The same strategy was applied to the synthesis of symmetrically fused cyclopropane-γ-lactams starting from allyldiazoacetamides. 148
Very recently, Arnold et al. evolved a hemoprotein (P411) that exhibits a mechanism entailing an N–H insertion and a protonation at the active site of the protein. This engineered active site also places the necessary water molecules for rapid and stereoselective proton rearrangement prior to product release. The ArM works in whole cells, exhibiting high activity and enantioselectivity (up to 98% ee). 149
All the above reactions are based on the modification of native metalloprotein systems. The intracellular assembly of metalloproteins equipped with non-natural metal cofactors is more difficult.
An interesting example from Brustad's group deals with the engineering of cytochrome P450 to selectively incorporate Ir(Me)–deuteroporphyrin IX (Ir(Me)–DPIX), in lieu of natural heme, directly in bacteria. The strategy required the introduction of mutations within the heme binding pocket. The resulting “in-cell” assembled iridium complex promoted olefin cyclopropanation reactions by EDA, and showed enhanced activity for aliphatic and electron-deficient olefins compared to the native heme enzyme. 150
Hartwig and coworkers recently demonstrated the viability of assembling an iridium containing heme metalloenzyme in bacteria, using the HUG system to transport the Ir(Me)MPIX cofactor into engineered cells expressing CYP119. They even demonstrated that it is possible to combine natural and artificial metalloenzymes in E. coli to afford cyclopropanated products from simple sugars ( Fig. 19 ). 151 Very recently, they identified a second system for transporting iridium porphyrin into Nissle 1917 (a non-pathogenic E. coli strain), based on the presence of an outer-membrane receptor of these cells. 152
Combination of microbial metabolism and artificial metalloenzyme cyclopropanation to generate cyclopropanes from D-glucose in E. coli reported by Hartwig (CYP119 PDB ID: ). |
In addition to carbene transfer reactions from diazo precursors, metal carbenes are also key reagents and intermediates in alkene metathesis processes, which can also be catalyzed by artificial metaloenzymes. 101 Ward and coworkers designed a biotinylated Hoveyda–Grubbs second-generation catalyst (biot-Ru) that binds to streptavidin in the periplasm of E. coli , to give an effective artificial metalloenzyme that promotes a ring-closing-metathesis ( Fig. 20 , left).
In vivo metathesis reactions developed by (a) Ward and (b) Tanaka. |
A similar approach, based on the affinity of coumarin for albumin, was employed by the group of Tanaka to build ArMs that perform “in cell” metathesis reactions ( Fig. 20 , right). 153
6. Conclusions
Considering the rich and versatile chemistry of metal carbenes, we foresee a bright future for further expanding the applications of these intermediates in chemical and cell biology, as well as biomedicine.
Current challenges include, among others, the creation of versatile artificial metalloenzymes that can perform chemoselective and bioorthogonal metal-carbene transfer reactions in mammalian cells, increasing rates and turnover with discrete metal catalysts, the development of metallocatalysts embedded in nanoscaffolds that facilitate the reactivity, or the development of biological applications.
Author contributions
Conflicts of interest, acknowledgements, notes and references.
- J. F. Hartwig, Organotransition metal chemistry: from bonding to catalysis , University Science Books: Mill Valley, CA, 2010 Search PubMed .
- P. H. Dixneuf and V. Cadierno, Metal-catalyzed reactions in water , Wiley-VCH Verlag GmbH & Co. KGaA, 2013 Search PubMed .
- S. Narayan, J. Muldoon, M. G. Finn, V. V. Fokin, H. C. Kolb and K. B. Sharpless, Angew. Chem., Int. Ed. , 2005, 44 , 3275–3279 CrossRef CAS PubMed .
- E. M. Sletten and C. R. Bertozzi, Angew. Chem., Int. Ed. , 2009, 48 , 6974–6998 CrossRef CAS PubMed .
- N. K. Devaraj, ACS Cent. Sci. , 2018, 4 , 952–959 CrossRef CAS PubMed .
- Y. Bai, J. Chen and S. C. Zimmerman, Chem. Soc. Rev. , 2018, 47 , 1811–1821 RSC .
- A. J. Link and D. A. Tirrell, J. Am. Chem. Soc. , 2003, 125 , 11164–11165 CrossRef CAS PubMed .
- A. J. Link, M. K. S. Vink and D. A. Tirrell, J. Am. Chem. Soc. , 2004, 126 , 10598–10602 CrossRef CAS PubMed .
- V. Hong, N. F. Steinmetz, M. Manchester and M. G. Finn, Bioconjugate Chem. , 2010, 21 , 1912–1916 CrossRef CAS PubMed .
- Y. Bai, X. Feng, H. Xing, Y. Xu, B. K. Kim, N. Baig, T. Zhou, A. A. Gewirth, Y. Lu, E. Oldfield and S. C. Zimmerman, J. Am. Chem. Soc. , 2016, 138 , 11077–11080 CrossRef CAS PubMed .
- J. Miguel-Ávila, M. Tomás-Gamasa, A. Olmos, P. J. Pérez and J. L. Mascareñas, Chem. Sci. , 2018, 9 , 1947–1952 RSC .
- C. Uttamapinant, M. I. Sanchez, D. S. Liu, J. Z. Yao, K. A. White, S. Grecian, S. Clark, K. R. Gee and A. Y. Ting, Nat. Protoc. , 2013, 8 , 1620–1634 CrossRef PubMed .
- L. Li and Z. Zhang, Molecules , 2016, 21 , 1393 CrossRef PubMed .
- C. Streu and E. Meggers, Angew. Chem., Int. Ed. , 2006, 45 , 5645–5648 CrossRef CAS PubMed .
- M. Martínez and J. L. Mascareñas, Coord. Chem. Rev. , 2018, 359 , 57–79 CrossRef .
- J. G. Rebelein and T. R. Ward, Curr. Opin. Biotechnol. , 2018, 53 , 106–114 CrossRef CAS PubMed .
- A. H. Ngo, S. Bose and L. H. Do, Chem.–Eur. J. , 2018, 24 , 10584–10594 CrossRef CAS PubMed .
- Y. Liu and Y. Bai, ACS Appl. Bio Mater. , 2020, 3 , 4717–4746 CrossRef CAS PubMed .
- P. Destito, C. Vidal, F. López and J. L. Mascareñas, Chem.–Eur. J. , 2021, 27 , 4789–4816 CrossRef CAS PubMed .
- T.-C. Chang and K. Tanaka, Bioorg. Med. Chem. , 2021, 46 , 116353 CrossRef CAS PubMed .
- J. J. Soldevila-Barreda and N. Metzler-Nolte, Chem. Rev. , 2019, 119 , 829–869 CrossRef CAS PubMed .
- M. O. N. van de L'Isle, M. C. Ortega-Liebana and A. Unciti-Broceta, Curr. Opin. Chem. Biol. , 2021, 61 , 32–42 CrossRef PubMed .
- W. Wanga, X. Zhang, R. Huang, C.-M. Hirschbiegel, H. Wang, Y. Ding and V. M. Rotello, Adv. Drug Delivery Rev. , 2021, 176 , 113893 CrossRef PubMed .
- S. Fedeli, J. Im, S. Gopalakrishnan, J. L. Elia, A. Gupta, D. Kimade and V. M. Rotello, Chem. Soc. Rev. , 2021, 50 , 13467–13480 RSC .
- P. K. Sasmal, S. Carregal-Romero, W. J. Parak and E. Meggers, Organometallics , 2012, 31 , 5968–5970 CrossRef CAS .
- M. I. Sánchez, C. Penas, M. E. Vázquez and J. L. Mascareñas, Chem. Sci. , 2014, 5 , 1901–1907 RSC .
- T. Volker, F. Dempwolff, P. L. Graumann and E. Meggers, Angew. Chem., Int. Ed. , 2014, 53 , 10536–10540 CrossRef PubMed .
- M. Tomás-Gamasa, M. Martínez-Calvo, J. R. Couceiro and J. L. Mascareñas, Nat. Commun. , 2016, 7 , 12538 CrossRef PubMed .
- G. Y. Tonga, Y. Jeong, B. Duncan, T. Mizuhara, R. Mout, R. Das, S. T. Kim, Y.-C. Yeh, B. Yan, S. Hou and V. M. Rotello, Nat. Chem. , 2015, 7 , 597–603 CrossRef CAS PubMed .
- M. Martínez-Calvo, J. R. Couceiro, P. Destito, J. Rodríguez, J. Mosquera and J. L. Mascareñas, ACS Catal. , 2018, 8 , 6055–6061 CrossRef PubMed .
- R. Das, R. F. Landis, G. Y. Tonga, R. Cao-Milan, D. C. Luther and V. M. Rotello, ACS Nano , 2019, 13 , 229–235 CrossRef CAS PubMed .
- S. Learte-Aymamí, C. Vidal, A. Gutiérrez-González and J. L. Mascareñas, Angew. Chem., Int. Ed. , 2020, 59 , 9149–9154 CrossRef PubMed .
- X. Zhang, S. Fedeli, S. Gopalakrishnan, R. Huang, A. Gupta, D. C. Luther and V. Rotello, ChemBioChem , 2020, 21 , 2759–2763 CrossRef CAS PubMed .
- P. Destito, J. R. Couceiro, H. Ferreira, F. López and J. L. Mascareñas, Angew. Chem., Int. Ed. , 2017, 56 , 10766–10770 CrossRef CAS PubMed .
- A. Gutiérrez-González, P. Destito, J. R. Couceiro, C. Pérez-González, F. López and J. L. Mascareñas, Angew. Chem., Int. Ed. , 2021, 60 , 16059–16066 CrossRef PubMed .
- J. Miguel-Ávila, M. Tomás-Gamasa and J. L. Mascareñas, Angew. Chem., Int. Ed. , 2020, 59 , 17628–17633 CrossRef PubMed .
- C. Vidal, M. Tomás-Gamasa, A. Gutiérrez-González and J. L. Mascareñas, J. Am. Chem. Soc. , 2019, 141 , 5125–5129 CrossRef CAS PubMed .
- C. Vidal, M. Tomás-Gamasa, P. Destito, F. López and J. L. Mascareñas, Nat. Commun. , 2018, 9 , 1913 CrossRef PubMed .
- N. Li, C. P. Ramil, R. K. V. Lim and Q. Lin, ACS Chem. Biol. , 2015, 10 , 379–384 CrossRef CAS PubMed .
- R. M. Yusop, A. Unciti-Broceta, E. M. V. Johansson, R. M. Sánchez-Martín and M. Bradley, Nat. Chem. , 2011, 3 , 239–243 CrossRef CAS PubMed .
- J. Clavadetscher, E. Indrigo, S. V. Chankeshwara, A. Lilienkampf and M. Bradley, Angew. Chem., Int. Ed. , 2017, 56 , 6864–6868 CrossRef CAS PubMed .
- L. Lercher, J. F. McGouran, B. M. Kessler, C. J. Schofield and B. G. Davis, Angew. Chem., Int. Ed. , 2013, 52 , 10553–10558 CrossRef CAS PubMed .
- P. Destito, A. Sousa-Castillo, J. R. Couceiro, F. López, M. A. Correa-Duarte and J. L. Mascareñas, Chem. Sci. , 2019, 10 , 2598–2603 RSC .
- J. Wang, C.-M. Che and M. P. Doyle, Transition Metal-Catalyzed Carbene Transformations , WILEY-VCH Verlag GmbH, 2021 Search PubMed .
- F. Zaragoza Dörwald, Metal carbenes in organic synthesis , WILEY-VCH Verlag GmbH, 1999 Search PubMed .
- P. de Frémont, N. Marion and S. P. Nolan, Coord. Chem. Rev. , 2009, 253 , 862–892 CrossRef .
- M. Jia and S. Ma, Angew. Chem., Int. Ed. , 2016, 55 , 9134–9166 CrossRef CAS PubMed .
- A. Ford, H. Miel, A. Ring, C. N. Slattery, A. R. Maguire and M. A. McKervey, Chem. Rev. , 2015, 115 , 9981–10080 CrossRef CAS PubMed .
- D. Zhu, J. Ma, K. Luo, H. Fu, L. Zhang and S. Zhu, Angew. Chem., Int. Ed. , 2016, 55 , 8452–8456 CrossRef CAS PubMed .
- H. M. L. Davies and R. E. J. Beckwith, Chem. Rev. , 2003, 103 , 2861–2903 CrossRef CAS PubMed .
- M. Brookhart and W. B. Studabaker, Chem. Rev. , 1987, 87 , 411–432 CrossRef CAS .
- D. Intrieri, A. Caselli and E. Gallo, Eur. J. Inorg. Chem. , 2011, 5071–5081 CrossRef CAS .
- H. M. L. Davies and E. G. Antoulinakis, Org. React. , 2001, 57 , 1–326 CAS .
- H. M. L. Davies and J. R. Manning, Nature , 2008, 451 , 417–424 CrossRef CAS PubMed .
- M. P. Doyle, R. Duffy, M. Ratnikov and L. Zhou, Chem. Rev. , 2010, 110 , 704–724 CrossRef CAS PubMed .
- M. Álvarez, R. Gava, M. R. Rodríguez, S. G. Rull and P. J. Pérez, ACS Catal. , 2017, 7 , 3707–3711 CrossRef .
- S.-F. Zhu and Q.-L. Zhou, Acc. Chem. Res. , 2012, 45 , 1365–1377 CrossRef CAS PubMed .
- D. Gillingham and N. Fei, Chem. Soc. Rev. , 2013, 42 , 4918–4931 RSC .
- W. Kirmse and M. Kapps, Chem. Ber. , 1968, 101 , 994–1003 CrossRef CAS .
- M. P. Doyle, J. H. Griffin, M. S. Chinn and D. van Leusen, J. Org. Chem. , 1984, 49 , 1917–1925 CrossRef CAS .
- S. Jana, Y. Guo and R. M. Koenigs, Chem.–Eur. J. , 2021, 27 , 1270–1281 CrossRef CAS PubMed .
- M. C. Pirrung, H. Liu and A. T. Morehead, J. Am. Chem. Soc. , 2002, 124 , 1014–1023 CrossRef CAS PubMed .
- For specific reviews on olefin metathesis in aqueous and biological environments, see: M. S. Messina and H. D. Maynard, Mater. Chem. Front. , 2020, 4 , 1040–1051 RSC ; J. B. Binder and R. T. Raines, Curr. Opin. Chem. Biol. , 2008, 12 , 767–773 CrossRef CAS PubMed .
- S. Iwasa, F. Takezawa, Y. Tuchiya and H. Nishiyama, Chem. Commun. , 2001, 59–60 RSC .
- R. P. Wurz and A. B. Charette, Org. Lett. , 2002, 4 , 4531–4533 CrossRef CAS PubMed .
- I. Nicolas, P. Le Maux and G. Simonneaux, Tetrahedron Lett. , 2008, 49 , 5793–5795 CrossRef CAS .
- M. Otte, P. F. Kuijpers, O. Troeppner, I. Ivanović-Burmazović, J. N. H. Reek and B. de Bruin, Chem.–Eur. J. , 2014, 20 , 4880–4884 CrossRef CAS PubMed .
- M. C. M. van Oers, L. K. E. A. Abdelmohsen, F. P. J. T. Rutjes and J. C. M. van Hest, Chem. Commun. , 2014, 50 , 4040–4043 RSC .
- N. R. Candeias, P. M. P. Gois and C. A. M. Afonso, Chem. Commun. , 2005, 391–393 RSC .
- N. R. Candeias, P. M. P. Gois and C. A. M. Afonso, J. Org. Chem. , 2006, 71 , 5489–5497 CrossRef CAS PubMed .
- L. Li, C. Shu, B. Zhou, Y.-F. Yu, X.-Y. Xiao and L.-W. Ye, Chem. Sci. , 2014, 5 , 4057–4064 RSC .
- H. F. Srour, P. Le Maux, S. Chevance, D. Carrié, N. Le Yondre and G. Simonneaux, J. Mol. Catal. A: Chem. , 2015, 407 , 194–203 CrossRef CAS .
- K. Ramakrishna, J. M. Thomas and C. Sivasankar, J. Org. Chem. , 2016, 81 , 9826–9835 CrossRef CAS PubMed .
- K. Ramakrishna and C. Sivasankar, Org. Biomol. Chem. , 2017, 15 , 2392–2396 RSC .
- R. P. Pandit, S. H. Kim and Y. R. Lee, Adv. Synth. Catal. , 2016, 358 , 3586–3599 CrossRef CAS .
- P. Saha, H. Jeon, P. K. Mishra, H.-W. Rhee and J. H. Kwak, J. Mol. Catal. A: Chem. , 2016, 417 , 10–18 CrossRef CAS .
- I. Aviv and Z. Gross, Chem.–Eur. J. , 2008, 14 , 3995–4005 CrossRef CAS PubMed .
- L. Zhou, Y. Liu, Y. Zhang and J. Wang, Beilstein J. Org. Chem. , 2011, 7 , 631–637 CrossRef CAS PubMed .
- M. Liao and J. Wang, Green Chem. , 2007, 9 , 184–188 RSC .
- T. G. Rajagopalan, W. H. Stein and S. Moore, J. Biol. Chem. , 1966, 241 , 4295–4297 CrossRef CAS PubMed .
- G. R. Delpierre and J. S. Fruton, Proc. Natl. Acad. Sci. U. S. A. , 1966, 1817–1822 CrossRef CAS PubMed .
- J. M. Antos and M. B. Francis, J. Am. Chem. Soc. , 2004, 126 , 10256–10257 CrossRef CAS PubMed .
- J. M. Antos, J. M. McFarland, A. T. Iavarone and M. B. Francis, J. Am. Chem. Soc. , 2009, 131 , 6301–6308 CrossRef CAS PubMed .
- R. Kundu and Z. T. Ball, Chem. Commun. , 2013, 49 , 4166–4168 RSC .
- C.-M. Ho, J.-L. Zhang, C.-Y. Zhou, O.-Y. Chan, J. J. Yan, F.-Y. Zhang, J.-S. Huang and C.-M. Che, J. Am. Chem. Soc. , 2010, 132 , 1886–1894 CrossRef CAS PubMed .
- M. Salmain, E. Licandro, C. Baldoli, S. Maiorana, H. Tran-Huy and G. Jaouen, J. Organomet. Chem. , 2001, 617–618 , 376–382 CrossRef CAS .
- P. Dutta, S. Sawoo, N. Ray, O. Bouloussa and A. Sarkar, Bioconjugate Chem. , 2011, 22 , 1202–1209 CrossRef CAS PubMed .
- B. V. Popp and Z. T. Ball, J. Am. Chem. Soc. , 2010, 132 , 6660–6662 CrossRef CAS PubMed .
- B. V. Popp and Z. T. Ball, Chem. Sci. , 2011, 2 , 690–695 RSC .
- Z. Chen, F. Vohidov, J. M. Coughlin, L. J. Stagg, S. T. Arold, J. E. Ladbury and Z. T. Ball, J. Am. Chem. Soc. , 2012, 134 , 10138–10145 CrossRef CAS PubMed .
- F. Vohidov, J. M. Coughlin and Z. T. Ball, Angew. Chem., Int. Ed. , 2015, 54 , 4587–4591 CrossRef CAS PubMed .
- J. Ohata and Z. T. Ball, J. Am. Chem. Soc. , 2017, 139 , 12617–12622 CrossRef CAS PubMed .
- K. Tishinov, K. Schmidt, D. Häussinger and D. G. Gillingham, Angew. Chem., Int. Ed. , 2012, 51 , 12000–12004 CrossRef CAS PubMed .
- K. Tishinov, N. Fei and D. Gillingham, Chem. Sci. , 2013, 4 , 4401–4406 RSC .
- S. N. Geigle, L. A. Wyss, S. J. Sturla and D. G. Gillingham, Chem. Sci. , 2017, 8 , 499–506 RSC .
- Y.-H. Lee, E. Yu and C.-M. Park, Nat. Commun. , 2021, 12 , 1681 CrossRef CAS PubMed .
- F. Schwizer, Y. Okamoto, T. Heinisch, Y. Gu, M. M. Pellizzoni, V. Lebrun, R. Reuter, V. Köhler, J. C. Lewis and T. R. Ward, Chem. Rev. , 2018, 118 , 142–231 CrossRef CAS PubMed .
- M. Diéguez, J.-E. Bäckvall and O. Pàmies, Artificial metalloenzymes and metalloDNAzymes in catalysis: from design to applications , Wiley-VCH. Weinheim, 2018 Search PubMed .
- M. Jeschek, S. Panke and T. R. Ward, Trends Biotechnol. , 2018, 36 , 60–72 CrossRef CAS PubMed .
- H. J. Davis and T. R. Ward, ACS Cent. Sci. , 2019, 5 , 1120–1136 CrossRef CAS PubMed .
- M. Jeschek, R. Reuter, T. Heinisch, C. Trindler, J. Klehr, S. Panke and T. R. Ward, Nature , 2016, 537 , 661–665 CrossRef CAS PubMed .
- M. Wittwer, U. Markel, J. Schiffels, J. Okuda, D. F. Sauer and U. Schwaneberg, Nat. Catal. , 2021, 4 , 814–827 CrossRef CAS .
- S. Chordia, S. Narasimhan, A. Lucini Paioni, M. Baldus and G. Roelfes, Angew. Chem., Int. Ed. , 2021, 60 , 5913–5920 CrossRef CAS PubMed .
- P. S. Coelho, E. M. Brustad, A. Kannan and F. H. Arnold, Science , 2013, 339 , 307–310 CrossRef CAS PubMed .
- P. S. Coelho, Z. J. Wang, M. E. Ener, S. A. Baril, A. Kannan, F. H. Arnold and E. M. Brustad, Nat. Chem. Biol. , 2013, 9 , 485–487 CrossRef CAS PubMed .
- H. Renata, Z. J. Wang, R. Z. Kitto and F. H. Arnold, Catal. Sci. Technol. , 2014, 4 , 3640–3643 RSC .
- K. E. Hernandez, H. Renata, R. D. Lewis, S. B. J. Kan, C. Zhang, J. Forte, D. Rozzell, J. A. McIntosh and F. H. Arnold, ACS Catal. , 2016, 6 , 7810–7813 CrossRef CAS PubMed .
- K. Chen and F. H. Arnold, J. Am. Chem. Soc. , 2020, 142 , 6891–6895 CrossRef CAS PubMed .
- Z. J. Wang, N. E. Peck, H. Renata and F. H. Arnold, Chem. Sci. , 2014, 5 , 598–601 RSC .
- S. B. J. Kan, R. D. Lewis, K. Chen and F. H. Arnold, Science , 2016, 354 , 1048–1051 CrossRef CAS PubMed .
- B. J. Kan, X. Huang, Y. Gumulya, K. Chen and F. H. Arnold, Nature , 2017, 552 , 132–136 CrossRef PubMed .
- J. Zhang, X. Huang, R. K. Zhang and F. H. Arnold, J. Am. Chem. Soc. , 2019, 141 , 9798–9802 CrossRef CAS PubMed .
- R. K. Zhang, K. Chen, X. Huang, L. Wohlschlager, H. Renata and F. H. Arnold, Nature , 2019, 565 , 67–72 CrossRef CAS PubMed .
- M. Bordeaux, R. Singh and R. Fasan, Bioorg. Med. Chem. , 2014, 22 , 5697–5704 CrossRef CAS PubMed .
- M. Bordeaux, V. Tyagi and R. Fasan, Angew. Chem., Int. Ed. , 2015, 54 , 1744–1748 CrossRef CAS PubMed .
- A. Tinoco, V. Steck, V. Tyagi and R. Fasan, J. Am. Chem. Soc. , 2017, 139 , 5293–5296 CrossRef CAS PubMed .
- A. L. Chandgude and R. Fasan, Angew. Chem., Int. Ed. , 2018, 57 , 15852–15856 CrossRef CAS PubMed .
- A. L. Chandgude, X. Ren and R. Fasan, J. Am. Chem. Soc. , 2019, 141 , 9145–9150 CrossRef PubMed .
- V. Steck, D. M. Carminati, N. R. Johnson and R. Fasan, ACS Catal. , 2020, 10 , 10967–10977 CrossRef CAS PubMed .
- D. Nam, V. Steck, R. J. Potenzino and R. Fasan, J. Am. Chem. Soc. , 2021, 143 , 2221–2231 CrossRef CAS PubMed .
- G. Sreenilayam and R. Fasan, Chem. Commun. , 2015, 51 , 1532–1534 RSC .
- V. Steck, G. Sreenilayam and R. Fasan, Synlett , 2020, 31 , 224–229 CrossRef CAS PubMed .
- V. Tyagi, R. B. Bonn and R. Fasan, Chem. Sci. , 2015, 6 , 2488–2494 RSC .
- R. L. Khade, A. L. Chandgude, R. Fasan and Y. Zhan, ChemCatChem , 2019, 11 , 3101–3108 CrossRef CAS PubMed .
- V. Tyagi, G. Sreenilayam, P. Bajaj, A. Tinoco and R. Fasan, Angew. Chem., Int. Ed. , 2016, 55 , 13563–13566 Search PubMed .
- H. M. Key, P. Dydio, D. S. Clark and J. F. Hartwig, Nature , 2016, 534 , 534–537 CrossRef CAS PubMed .
- P. Dydio, H. M. Key, A. Nazarenko, J. Y.-E. Rha, V. Seyedkazemi, D. S. Clark and J. F. Hartwig, Science , 2016, 354 , 102–106 CrossRef CAS PubMed .
- Y. Gu, S. N. Natoli, Z. Liu, D. S. Clark and J. F. Hartwig, Angew. Chem., Int. Ed. , 2019, 58 , 13954–13960 CrossRef CAS PubMed .
- M. W. Wolf, D. A. Vargas and N. Lehnert, Inorg. Chem. , 2017, 56 , 5623–5635 CrossRef CAS PubMed .
- K. Oohora, H. Meichin, L. Zhao, M. W. Wolf, A. Nakayama, J. Hasegawa, N. Lehnert and T. Hayashi, J. Am. Chem. Soc. , 2017, 139 , 17265–17268 CrossRef CAS PubMed .
- H. Yang, P. Srivastava, C. Zhang and J. C. Lewis, ChemBioChem , 2014, 15 , 223–227 CrossRef CAS PubMed .
- P. Srivastava, H. Yang, K. Ellis-Guardiola and J. C. Lewis, Nat. Commun. , 2015, 6 , 7789 CrossRef CAS PubMed .
- D. M. Upp, R. Huang, Y. Li, M. J. Bultman, B. Roux and J. C. Lewis, Angew. Chem., Int. Ed. , 2021, 60 , 23672–23677 CrossRef CAS PubMed .
- L. Villarino, K. E. Splan, E. Reddem, L. Alonso-Cotchico, C. Gutiérrez de Souza, A. Lledós, J.-D. Maréchal, A.-M. W. H. Thunnissen and G. Roelfes, Angew. Chem., Int. Ed. , 2018, 57 , 7785–7789 CrossRef PubMed .
- M. E. Wilson and G. M. Whitesides, J. Am. Chem. Soc. , 1978, 100 , 306–307 CrossRef CAS .
- J. Zhao, D. G. Bachmann, M. Lenz, D. G. Gillingham and T. R. Ward, Catal. Sci. Technol. , 2018, 8 , 2294–2298 RSC .
- J. Oelerich and G. Roelfes, Chem. Sci. , 2013, 4 , 2013–2017 RSC .
- A. Rioz-Martínez, J. Oelerich, N. Ségaud and G. Roelfes, Angew. Chem., Int. Ed. , 2016, 55 , 14136–14140 CrossRef PubMed .
- H. Ibrahim, P. Mulyk and D. Sen, ACS Omega , 2019, 4 , 15280–15288 CrossRef CAS PubMed .
- J. Hao, W. Miao, Y. Cheng, S. Lu, G. Jia and C. Li, ACS Catal. , 2020, 10 , 6561–6567 CrossRef CAS .
- L. Adriaenssens, L. Severa, J. Vávra, T. Šálová, J. Hývl, M. Čížková, R. Pohl, D. Šaman and F. Teplý, Collect. Czech. Chem. Commun. , 2009, 74 , 1023–1034 CrossRef CAS .
- S. Wallace and E. P. Balskus, Angew. Chem., Int. Ed. , 2015, 54 , 7106–7109 CrossRef CAS PubMed .
- S. Gutiérrez, M. Tomás-Gamasa and J. L. Mascareñas, Angew. Chem., Int. Ed. , 2021, 60 , 22017–22025 CrossRef PubMed .
- M. Garcia-Borràs, S. B. J. Kan, R. D. Lewis, A. Tang, G. Jimenez-Osés, F. H. Arnold and K. N. Houk, J. Am. Chem. Soc. , 2021, 143 , 7114–7123 CrossRef PubMed .
- K. Chen, X. Huang, S. B. J. Kan, R. K. Zhang and F. H. Arnold, Science , 2018, 360 , 71–75 CrossRef CAS PubMed .
- X. Ren, A. L. Chandgude and R. Fasan, ACS Catal. , 2020, 10 , 2308–2313 CrossRef CAS PubMed .
- Z. Liu, C. Calvó-Tusell, A. Z. Zhou, K. Chen, M. García-Borràs and F. H. Arnold, Nat. Chem. , 2021, 13 , 1166–1172 CrossRef CAS PubMed .
- E. W. Reynolds, T. D. Schwochert, M. W. McHenry, J. W. Watters and E. M. Brustad, ChemBioChem , 2017, 18 , 2380–2384 CrossRef CAS PubMed .
- J. Huang, Z. Liu, B. J. Bloomer, D. S. Clark, A. Mukhopadhyay, J. D. Keasling and J. F. Hartwig, Nat. Chem. , 2021, 13 , 1186–1191 CrossRef CAS PubMed .
- Z. Liu, J. Huang, Y. Gu, D. S. Clark, A. Mukhopadhyay, J. D. Keasling and J. F. Hartwig, J. Am. Chem. Soc. , 2022, 144 , 883–890 CrossRef CAS PubMed .
- I. Nasibullin, I. Smirnov, P. Ahmadi, K. Vong, A. Kurbangalieva and K. Tanaka, Nat. Commun. , 2022, 13 , 39 CrossRef CAS PubMed .

An official website of the United States government
The .gov means it’s official. Federal government websites often end in .gov or .mil. Before sharing sensitive information, make sure you’re on a federal government site.
The site is secure. The https:// ensures that you are connecting to the official website and that any information you provide is encrypted and transmitted securely.
- Publications
- Account settings
- My Bibliography
- Collections
- Citation manager
Save citation to file
Email citation, add to collections.
- Create a new collection
- Add to an existing collection
Add to My Bibliography
Your saved search, create a file for external citation management software, your rss feed.
- Search in PubMed
- Search in NLM Catalog
- Add to Search
Recent progress in the development of organometallics for the treatment of cancer
Affiliations.
- 1 Department of Chemistry and Biochemistry, University of Hull, Cottingham Road, Hull HU6 7RX, United Kingdom. Electronic address: [email protected].
- 2 Institut des Sciences et Ingénierie Chimiques (ISIC), Ecole Polytechnique Fédérale de Lausanne (EPFL), 1015 Lausanne, Switzerland. Electronic address: [email protected].
- PMID: 31812831
- DOI: 10.1016/j.cbpa.2019.11.001
From their early successes in medicine, organometallic compounds continue to attract interest as potential chemotherapeutics to treat a range of diseases. Here, we show from recent literature selected largely from the last two years that organometallics offer unique opportunities in medicine and, increasingly, a mechanistic-based approach is applied to their development, which has not always been the case.
Keywords: Bioinorganic chemistry; Bioorganometallic chemistry; Chemotherapy; Metallodrugs.
Copyright © 2019 Elsevier Ltd. All rights reserved.
PubMed Disclaimer
Conflict of interest statement
Conflict of interest The authors declare no conflict of interest.
Similar articles
- Ruthenium versus platinum: interactions of anticancer metallodrugs with duplex oligonucleotides characterised by electrospray ionisation mass spectrometry. Groessl M, Tsybin YO, Hartinger CG, Keppler BK, Dyson PJ. Groessl M, et al. J Biol Inorg Chem. 2010 Jun;15(5):677-88. doi: 10.1007/s00775-010-0635-0. Epub 2010 Mar 7. J Biol Inorg Chem. 2010. PMID: 20213306 Free PMC article.
- Platinum, palladium, gold and ruthenium complexes as anticancer agents: Current clinical uses, cytotoxicity studies and future perspectives. Lazarević T, Rilak A, Bugarčić ŽD. Lazarević T, et al. Eur J Med Chem. 2017 Dec 15;142:8-31. doi: 10.1016/j.ejmech.2017.04.007. Epub 2017 Apr 18. Eur J Med Chem. 2017. PMID: 28442170 Review.
- Light-activated ruthenium complexes photobind DNA and are cytotoxic in the photodynamic therapy window. Wachter E, Heidary DK, Howerton BS, Parkin S, Glazer EC. Wachter E, et al. Chem Commun (Camb). 2012 Oct 7;48(77):9649-51. doi: 10.1039/c2cc33359g. Chem Commun (Camb). 2012. PMID: 22908094
- Structure-activity relationships for ruthenium and osmium anticancer agents - towards clinical development. Meier-Menches SM , Gerner C , Berger W , Hartinger CG , Keppler BK . Meier-Menches SM , et al. Chem Soc Rev. 2018 Feb 5;47(3):909-928. doi: 10.1039/c7cs00332c. Chem Soc Rev. 2018. PMID: 29170783 Review.
- Exploring the activity of a polyazine bridged Ru(ii)-Pt(ii) supramolecule in F98 rat malignant glioma cells. Zhu J, Rodríguez-Corrales JÁ, Prussin R, Zhao Z, Dominijanni A, Hopkins SL, Winkel BS, Robertson JL, Brewer KJ. Zhu J, et al. Chem Commun (Camb). 2016 Dec 20;53(1):145-148. doi: 10.1039/c6cc07978d. Chem Commun (Camb). 2016. PMID: 27901157
- Unsymmetrical Pd(II) Pincer Complexes with Benzothiazole and Thiocarbamate Flanking Units: Expedient Solvent-Free Synthesis and Anticancer Potential. Kozlov VA, Aleksanyan DV, Churusova SG, Spiridonov AA, Rybalkina EY, Gutsul EI, Aksenova SA, Korlyukov AA, Peregudov AS, Klemenkova ZS. Kozlov VA, et al. Int J Mol Sci. 2023 Dec 10;24(24):17331. doi: 10.3390/ijms242417331. Int J Mol Sci. 2023. PMID: 38139160 Free PMC article.
- Effects of Ferrocene and Ferrocenium on MCF-7 Breast Cancer Cells and Interconnection with Regulated Cell Death Pathways. Favaron C, Gabano E, Zanellato I, Gaiaschi L, Casali C, Bottone MG, Ravera M. Favaron C, et al. Molecules. 2023 Sep 6;28(18):6469. doi: 10.3390/molecules28186469. Molecules. 2023. PMID: 37764244 Free PMC article.
- Anticancer Potential of Diruthenium Complexes with Bridging Hydrocarbyl Ligands from Bioactive Alkynols. Bresciani G, Vančo J, Funaioli T, Zacchini S, Malina T, Pampaloni G, Dvořák Z, Trávníček Z, Marchetti F. Bresciani G, et al. Inorg Chem. 2023 Oct 2;62(39):15875-15890. doi: 10.1021/acs.inorgchem.3c01731. Epub 2023 Sep 15. Inorg Chem. 2023. PMID: 37713240 Free PMC article.
- Biodegradable Grubbs-Loaded Artificial Organelles for Endosomal Ring-Closing Metathesis. Oerlemans RAJF, Shao J, van Stevendaal MHME, Wu H, Patiño Padial T, Abdelmohsen LKEA, van Hest JCM. Oerlemans RAJF, et al. Biomacromolecules. 2023 Sep 11;24(9):4148-4155. doi: 10.1021/acs.biomac.3c00487. Epub 2023 Aug 17. Biomacromolecules. 2023. PMID: 37589683 Free PMC article.
- Adding Diversity to a Diruthenium Biscyclopentadienyl Scaffold via Alkyne Incorporation: Synthesis and Biological Studies. Bresciani G, Boni S, Funaioli T, Zacchini S, Pampaloni G, Busto N, Biver T, Marchetti F. Bresciani G, et al. Inorg Chem. 2023 Aug 7;62(31):12453-12467. doi: 10.1021/acs.inorgchem.3c01644. Epub 2023 Jul 21. Inorg Chem. 2023. PMID: 37478132 Free PMC article.
Publication types
- Search in MeSH
Related information
- PubChem Compound (MeSH Keyword)
LinkOut - more resources
Full text sources.
- Elsevier Science
- Citation Manager
NCBI Literature Resources
MeSH PMC Bookshelf Disclaimer
The PubMed wordmark and PubMed logo are registered trademarks of the U.S. Department of Health and Human Services (HHS). Unauthorized use of these marks is strictly prohibited.

IMAGES
VIDEO
COMMENTS
Organometallic chemistry is the study of the synthesis, structure and reactivity of chemical compounds that contain metal-carbon bonds. These compounds are often used as homogeneous catalysts.
These requirements form the basis for performing thorough research on organometallic compounds, covering their production, characteristics, effects on the environment, and useful uses. This review article focuses on the various aspects of introduction of organometallic compounds.
The construction of carbon–carbon bonds is integral to the synthesis of organic molecules, and transition metal catalysts have excelled in accomplishing this task. The strength (bond dissociation energy) of a transition metal–carbon bond is substantially weaker than that of a carbon–carbon σ bond. The lability of metal–ligand ...
This Editorial and its accompanying collection of recently published articles point to current trends in the landscape of applied organometallic chemistry, establishing a role for organometallic compounds in emerging areas of research, including sustainable energy conversion/storage, medicinal chemistry/drug development, catalysis, and materials...
The use of organometallic compounds in organic chemistry is one of the cornerstones of the modern synthetic methodology for the activation and generation of new bonds in a molecule.
Studies in organometallic chemistry in CCB involve the design of new transition metal complexes that display specific, targeted functionality such as electron-transfer activity, redox behavior, or variable metal-metal interactions. Such complexes can function as models for biological metal-containing sites or as industrial catalysts, as examples.
The reliable catalytic methods of today were built on a solid foundation of organometallic chemistry, uncovering new reactivity modes and ultimately changing how synthetic organic chemists think about making molecules.
The CuAAC entails a typical organometallic mechanism involving oxidative cyclometallation and reductive elimination steps, and it is a fundamental reference in the field of biological organometallic catalysis.
Here, we show from recent literature selected largely from the last two years that organometallics offer unique opportunities in medicine and, increasingly, a mechanistic-based approach is applied to their development, which has not always been the case.
This chapter describes the basics of organometallic chemistry for readers relatively new to the subject. Organometallic chemistry is the field of chemistry that was born and grew up between metal complex chemistry and organic chemistry. It thus incorporates aspects from both ways of thinking.